Images of exclusion zone (“e-zone”) in this section are used courtesy of Prof. Gerald Pollack.
Exclusion zone water
Water is essential to the energy needs of the body because it stores energy from our surroundings, and later releases that energy to power cellular processes. That makes water a battery, and the sun our best battery charger. Without water, most bodily functions don’t work properly.
To give you some perspective on the importance of water, biochemists have traditionally studied biology by looking at its composition. Meaning, they take a cell or a tissue, break it down, empty out the water, and study it in a dehydrated state. But how much can you really know about how a cell works when you remove the single most important driver of cell function?
Water is an inseparable sculptor of health or sickness because it powers, contains, or otherwise facilitates the physics and chemistry of the body. More broadly speaking, you can take the cell out of the sea, but you can’t take the sea out of the cell. Without a doubt, there’s much more to water than you might think. But supporting the body’s biochemistry is only the beginning.
Only recently has science come to learn most of the work in the human body is done by water’s biophysical properties we never knew it had. More precisely, who would have thought at the turn of the century that water’s most important contribution to human health comes from its electrical potential?
The fourth phase of water
Defined scientifically by Professor Gerald Pollack and colleagues (based on the concepts of Gilbert Ling, Albert Szent-Györgyi, Walter Drost-Hansen and James Clegg), the fourth phase of water is one of the most intriguing scientific discoveries made in the last century, because it explains dozens of natural phenomena that happen around us every day, but science and medicine had yet to explain.
Its applications in science and medicine boggle the mind, because it defines a distinct phase of water between a liquid and a solid. It’s found almost everywhere in nature that normal water is present. And it has a unique structure, as well as its own remarkable properties.
Pollack dubbed the fourth phase of water “exclusion zone” water. But it goes by so many different names today – EZ water, charge-separated water, structured water, gel-state water, interfacial water, liquid crystalline water or H3O2 – that this newly-defined phase of water needs and deserves its own name. Let’s call it “e-zone,” for short.
It’s explained in these monographs because e-zone and zeta potential (net negative charge around particles) are partners in maintaining adequate charge in the blood, vessels, cells, and tissues. Strength of charge affects the hydrodynamic properties of the blood. Vessels depend on electrostatic charge to lubricate the endothelium against friction. And cells use electrical charge to power their processes.
However, cutting-edge researchers debate how much of each is present inside the cell and around red blood cells, as examples. Is the gel found in cells purely e-zone, or is it a classic polymer type of gel? Cells’ interiors certainly aren’t filled with pure water. And, if cells are filled with liquid crystalline water, what is its purpose? Great questions that biophysicists are still unpacking.
Electrical charge is Nature’s favorite force
To give you some background, most activity in the world around us – including that in the realms of biology, hi-tech, physics, and geology – revolves around electrical charge, because most work in the universe is done by ‘like’ charges repelling each other, or unlike charges attracting each other.
Gravity, in contrast, is many orders of magnitude weaker at making things move (by a factor of 1038). Positive and negative charges are the driving force that moves things in biological systems, in our man-made devices, and in geology. Nature uses electrical charge in so many ways because electrostatic attraction and repulsion is astonishingly strong, particularly at the subatomic level.
To illustrate, suppose you could collect the electrons flowing through each of two (lit) incandescent light bulbs for one second. Position those negative charges a few inches apart from each other, and how much repulsive force do you think they’d have? Try the weight of 50,000 garbage trucks. Now remove 1% of the electrons from each of two people, and those electrons placed a foot apart would repel each other with the force equal to the weight of the earth.
How water’s positive charge separates from its negative charge
Regular water (H2O) is composed of two hydrogen atoms, each of which has a +1 valence electrical charge, and one oxygen atom, which has a -2 valence electrical charge. When combined, this gives water a neutral electrical charge (1+1 of hydrogen – 2 of oxygen = 0). Hence, water’s neutral electrical charge can’t do any work (attract or repel) in its normal state. Yet those charges do contain tremendous energy potential, as we shall see.
Exclusion zone water is able to power cellular processes (i.e., chemical reactions and movement) by separating positively-charged hydrogen atoms (H) from negatively-charged OH groups, and rearranging them into hexagonal sheets, with a pool of those hydrogens beside it. Separate pools of charged particles thus create the electrical equivalent of electrolyte (the liquid with all the juice in a car’s lead-acid battery).
What happens is, around surfaces (especially water-loving surfaces), water naturally rearranges its atoms into layers of a honeycomb shape, which are one atom thick.
The polarized layers build up on top of each other, shifted sideways by one atom (i.e., oxygens in one layer are lined up with hydrogens from another). This forms a crystal lattice that’s easy to break apart due to its weak bonds.
These configurations, like a game of musical chairs, don’t have room for some of the hydrogen atoms in its hexagonal matrix. So the e-zone kicks the orphaned hydrogens into the surrounding space. These hydrogen atoms, and their positive charge, collect in a pool next to the e-zone layer, which is called “bulk water” or “unstructured water.” Thus, the layers of e-zone accumulate negative charge, while the water around the e-zone concentrates protons and positive charge. Violà, you have a battery.
Configurations like this are called liquid crystals because of their honeycomb-ordered structure (crystal) and weak bonds (viscous fluid).
In contrast, ice uses the same hexagonal structure as e-zone, only the layers are stacked directly on top of each other. The negatively-charged oxygens in one layer are bound to oxygens in the adjacent layer through the positively-charged loner hydrogen we just talked about, thus forming a strong, solid bond. This strong electrostatic connection is what makes ice solid.
Interesting to note, water goes through the e-zone phase before it turns into ice. And when ice melts, it becomes e-zone briefly before it turns back into regular water. For these reasons, sailors have noted for centuries that water surrounding icebergs is more viscous than regular water. The liquid crystal properties of e-zone explain why ships slow down as if sailing through Jell-O.
But the difference-maker for biology is that the size and strength of the exclusion zone grows when it’s exposed to light – particularly IR – thus separating more charge, storing more energy, and making a bigger battery. The wavelength of light that is best at building e-zone is infrared, followed by visible frequencies. This is how water stores the sun’s energy for our cells to use. And it’s how the circulatory system gains electrical charge around its surfaces and in its fluids.
How the heart and the blood really operate
Read the full version of this section in The Mitochondriac Manifesto.
How can the heart push individual blood cells through the body’s many miles of tiny capillaries, considering the fact that red blood cells (RBCs), 6–7 micrometers (µm) in diameter, are actually larger than the smallest capillaries they pass through (3–5 µm)? That means RBCs have to be deformed into an elliptical shape to fit through the narrowest capillaries.
Those two factors – distance and deformation – would create unimaginable resistance to fluid flow. Can you imagine the friction? Can you imagine the hydraulic pressure you’d need to push a viscous liquid through miles of microscopic tubes AND distort the RBCs at the far reaches of their circulation? Not to mention the pressure needed for the return trip.
It would require hydraulic pressure orders of magnitude greater than the heart can possibly produce and the vessels can withstand. So that line of thinking clearly does not hold water. Just try blowing air, which produces far less friction than water, through a straw more than ten feet long. You’ll see what I mean. Now try pushing water through rubber tubing a millimeter in diameter, 3,000 feet long, and clogged at the end.
That leads us to only one conclusion: There has to be more to blood flow than meets the eye. The heart can’t possibly do what we think it does. Instead, blood flow must be driven by other forces to overcome the unimaginable resistance that would theoretically be present, if blood flow worked the way people thought it did.
Thus, healthy blood flow is a combined effort of: (1) the electrostatic repulsion and attraction of charged particles that cancel friction and interconnect the blood; (2) the heart making blood flow pulsate and vortex; (3) the suction effect of the heart’s magnetism; and (4) electrical charge expanding vessels to then pump blood upon relaxation.
When the circulatory system receives contribution from each of its forces, blood flows around the body with minimal effort/maximum conservation of energy. But when these forces weaken due to disease, or when adjusting to exertion or temperature, the body compensates so the right amount of oxygen is delivered and waste evacuated.
The blood behaves as a single organ, with lots of roving parts
It’s tempting to think of the blood as being little more than a collection of cells in water. But Nature designed it to function more like a liquid-solid system, with trillions of pieces distributed throughout the body – all adapting to their local terrain, but working as one, courtesy of polarity. Blood, to put it simply, is not just a variety of cells floating in liquid. It is exquisitely engineered bio-machinery whose movement is more coordinated and compliant to vessel structure than its material or chemical properties suggest.
Counter-intuitively, total momentum of blood in the circulatory system does not change much from one point to the next. Its toughest challenge is probably to maintain some momentum before vessels branch into 3–5 micrometer capillaries… not just because the vessels are smaller than RBCs (certainly part of it) but, rather, the capillary bed is so spread out that individual cells each carry negligible momentum when they need it most: through the tiniest capillaries.
That’s why the body often resorts to raising blood pressure when the blood loses polarity and cohesiveness, and/or when the heart is not pulsing and vortexing the blood properly: cells need a minimal amount of momentum to keep flowing in the right direction and not stall somewhere midstream. Constricting the arteries to increase blood pressure is how the body usually hikes circulation to meet this ‘force of flow’ requirement. However, this has consequences.
We need to rethink our understanding of blood flow
Key to knowing how the circulatory systems works, blood is not pumped around the body by mechanical forces of high pressure pushing it into areas of low pressure. Rather, electrostatic repulsion and attraction do most of the work turning many individual cells into a marching band with many moves. Polarity makes the blood’s journey around the body virtually frictionless. Here’s how I came to this incredible realization:
In watching real video footage of how red blood cells actually move through small vessels, it was clear to me that areas of high pressure and low pressure could not possibly make the cells move the way that they do. Blood moves through the vessels too freely and homogenously (as-one) for that to be the case.
First conundrum: How do red blood cells get into the smallest capillaries? Why do capillaries less than one cell width in diameter get any RBCs to flow through them at all, when nearby vessels are far bigger, and offer far less resistance? You’d think blood cells would avoid the hassle of having to contort themselves when larger vessels offer a speedier, more comfortable ride, with no distortion needed. Doesn’t make any sense.
Second problem: How does the heart generate enough pressure to push red blood cells through cramped capillaries? The heart is too far away, and blood flow too widely distributed, for this passage to happen against the friction we would expect.
Third, how do red blood cells exit the smallest capillaries and rejoin their co-workers in line? RBCs with a normal zeta potential are supposed to repel each other with negative charge. But I saw very slow-moving RBCs leaving a capillary and entering a perpendicular, extremely fast-moving larger vessel that was completely packed with cells. There seemed to be no room to enter the fray, so they should have been blocked from entering by the repulsion of traffic flying by.
But the exact opposite was happening. After an instant of hesitation, there appeared to be some mysterious force violently yanking the RBCs into the smallest gaps in the flow. Something is grabbing them and pulling them into rapid circulation, which isn’t simply the force of suction. Judging by the way they get stretched out like a spaceship entering warp speed, the force couldn’t be mechanical suction; it has to be electrostatic.
Watch micro blood flow in these YouTube videos: video 1, video 2.
For blood to flow effortlessly, first you need to cancel friction
This is accomplished with zeta potential. Zeta potential is often described as making red blood cells slippery so they don’t clump together. That’s good enough for common consumers, but an even more exact understanding will greatly improve our ability to diagnose and treat cardiovascular disease. In my informal estimation, zeta potential is probably the first thing you want to fix when your cardiovascular system struggles.
Image from “Heart Disease: A New Approach to Prevention and Control” by Thomas M. Riddick, 1970.
Dark field image of red blood cells and one white blood cell. Note the RBCs look like they’re glowing. This is a dual layer of electrical charge, called zeta potential, which tends to include some materials such as water, while excluding others.
When RBCs lose electrical charge, the stickiness of their glycoproteins (glyco = sugar) promotes clumping (rouleaux formation) and clotting (emboli).
Platelets can stick together too (center cluster).
Zeta potential is actually a net-negative cloud of charged particles around red blood cells that encourage them to stay uniformly spaced from each another – meaning their round sides are very close together or touching, but their surfaces are not smushed together or very far apart. Blood cells are neither intimate partners nor strangers; they’re good friends with all their cell-mates. Conversely, when RBCs are low on zeta potential their concave faces stick together like a stack of coins, because RBCs are made of sticky glycoproteins. That’s incredibly bad for your circulation, oxygenation, and organ function.
Cells of the inner vessel wall (endothelium) also have a negative charge. So repulsive force is what propels net negative RBCs through negatively-charged blood vessels like a maglev train’s levitation and propulsion system. The net negative charge of zeta potential cancels friction between blood cells and the vessel walls – especially important in one-cell capillaries.
At the same time, protons in the serum give blood cohesiveness
Essential to blood’s interconnectedness, particles of positive charge in the serum (mostly from charge-separated water) are the attractive force that gives whole blood its urge for solidarity. Protons pull RBCs along when they need to accelerate – much more than mechanical force of suction does. Wherever the serum goes (blood’s liquid), it sucks the RBCs along, using positive-negative attraction. Imagine tiny magnets in the serum pulling RBCs through the smallest tunnels, against the greatest resistance, or merely into gaps in arteriole (small artery) flow.
Electrostatic attraction is as important as repulsion to blood flow:
- This explains a more plausible means by which RBCs get pulled through the narrowest capillaries, other than pressure differentials.
- This explains why small gaps in a larger, fast-flowing arteriole violently suck stationary RBCs into the flow from a single-file capillary: when red cells are spaced out in a small capillary (i.e., more positive fluid, fewer negative cells), the net negative charge in a crowded arteriole (lots of RBCs) pulls the positive chargeof the serum into the flow and the red cells follow. RBCs in the capillary are pulled into the current like yanking a big water balloon out of someone’s hand.
- An absence of attraction explains the occurrence of low “ejection fractions” (the heart’s pumping efficiency): attraction between the liquid and the cells is low, so blood flowdoesn’t have its usual oneness. Each contraction then leaves more blood in the chamber.
- Tissue polarity is another way that blood manages to get through the capillary bed without losing much momentum. That is, oxygen-poor tissue in the capillary bed, which is positive, literally pushes proton-rich serum out of the way, while pulling oxygen-rich (negative) RBCs toward it. RBCs are linked to the serum like a chain of rubber bands.
- And this explains why, when you actually watch it, blood flowappears incredibly agile and energetic, as if red blood cells enthusiastically go wherever they’re needed, instead of fighting friction and momentum, were it not for electrostatics.
Now can you appreciate how RBCs like to keep some personal space and, at the same time, the positive charge in the serum keeps whole blood moving in a train-like fashion? Can you see why, except for when the heart interrupts the flow to create pulsation, all the blood in the entire circulatory system does its best to move in unison?
Gerald Pollack described this cohesion action in his book The Fourth Phase of Water. He wrote that objects of like charge in a solution are attracted to each other because their clouds of opposite charge surrounding each object comingle, pulling the objects together due to a doubling of electrostatic attraction between them (see image above). When/if the objects touch, the particles of opposite charge then glue the objects together with “like-opposite-like” charges. This explains why “like likes like” in physics.
Wet sand clings together using this phenomenon (unlike dry sand) to make sand castles, as do water droplets to form clouds, among many more scenarios. Well, the cells in whole blood express the very same dynamics, only the negative charge around RBCs is not a single layer of charged particles; it’s a zone composed of two layers. And blood is not fixed; it’s fluid.
The heart has 6 main jobs (none are pumping the blood)
- Makes blood flow pulsate. The heart stops blood flow for a split second to build a pressure differential between the supply side of the tricuspid valve and the delivery side. When the valve opens, a pressure wave is launched into the preceding one.
- Vortexes. It converts a laminar (flat) flow into a vortex(swirling).
- One-way valve. It keeps blood flowing in the right direction.
- Pulse rate. When you need more oxygen, the autonomic nervous system makes the heartbeat faster to increase the frequency of its pressure waves. This is how the heart regulates quantity of blood flow.
- Adds propulsion with the blood’s paramagnetism.
- Structures water molecules in the blood. The heart’s magnetic field structures the blood’s water molecules into smaller clusters. This enhances charge-separation and erases energetic residues.
The heart controls the quality of blood flow first and its quantity second. The heart shapes the way the blood flows, which is a large reason why blood is able to circulate around the body at all. If the circulatory system were not designed around Nature’s principles of implosion, acceleration, anti-friction, polarity, and conservation of energy – then the blood would not get very far, and complex life forms would not exist.
Watch the documentary “Secret of Water” on Gaia, or other streaming platforms, to learn more about water’s memory and restructuring it. It was omitted here for space considerations.
The heart operates similar to a hydraulic ram pump
Hydraulic ram pumps (aka “water hammer” pumps) have been used for centuries to move water uphill, using pressure differentials created by a flow of water. Ram pumps take in water from a fast-flowing stream (C) into a pipe (H) with a pressure accumulator (D). Water pressure slams a one-way valve shut (A), which causes positive pressure to build up on the supply side (H) of the one-way valve (G), which sucks water into the delivery side (B) of the valve (G). When valve (G) closes in the second half of the cycle, water is pushed through the system by the air pressure in accumulator (D). In this way, the kinetic energy of flowing water (C) is converted into pressure, which is used to move materials against gravity or resistance (E).
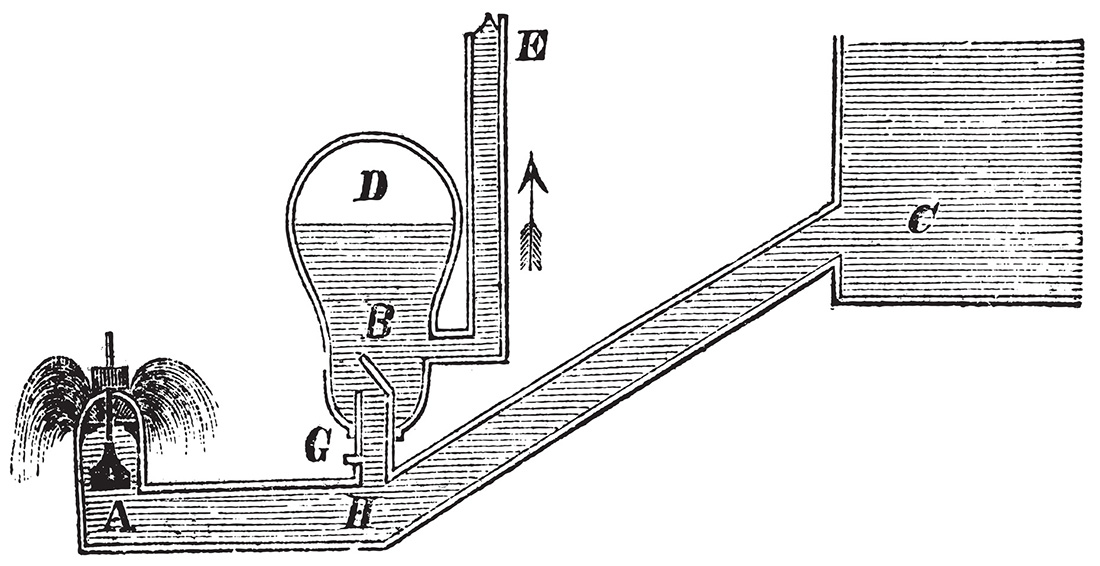
How a hydraulic ram pump operates: Water from source ‘C’ causes one-way valve ‘A’ to close. Water pressure increases around ‘H’, which pushes water through valve ‘G’ into vessel ‘B’, while the air contained in ‘D’ gets compressed. Soon, valve ‘G’ closes from the high pressure in ‘D’, which pushes water up pipe ‘E’. Meanwhile, valve ‘A’ has opened from the low pressure around ‘H’. That’s one full cycle.
See a hydraulic ram pump operating in the YouTube video entitled “How the ram pump works”.
The cardiovascular system uses a similar concept to help regulate blood flow, except in biology it’s a completely closed system, the components are in-line, and there’s no waste overflow. Instead, artery expansion is the accumulator. The heart is essentially a modified hydraulic ram pump. In fact, hydraulic ram pumps are 70% efficient at best, while a healthy ejection fraction (a measurement of heart efficiency) for a fit person is also around 70%. Coincidence? I think not.
Furthermore, the blood is already moving at full speed when it reaches the heart. It leaves at that same speed, slows down each time the system branches, then picks up steam on its way back to the heart. But, crucial to our understanding, blood is motivated to move through the system by other means, which are described below. On the other hand, if the heart did all of the work pumping blood around the body, its pressure and velocity would be lowest before entering the heart, and highest when it leaves. But it’s not.
The heart makes blood flow pulsate and spiral
The tricuspid valve in the heart stops blood flow for a fraction of a section. This causes positive pressure to build up on the supply side of the valve, which expands the vessels (superior and inferior vena cava) with blood, while a vacuum builds up on the delivery side of the tricuspid (the right ventricle). When the tricuspid opens, the blood is both pushed and pulled out of the heart, which vortexes, cools, condenses, and structures its constituents. The left side of the heart structures blood flow the same way, along with oxygen from the lungs.
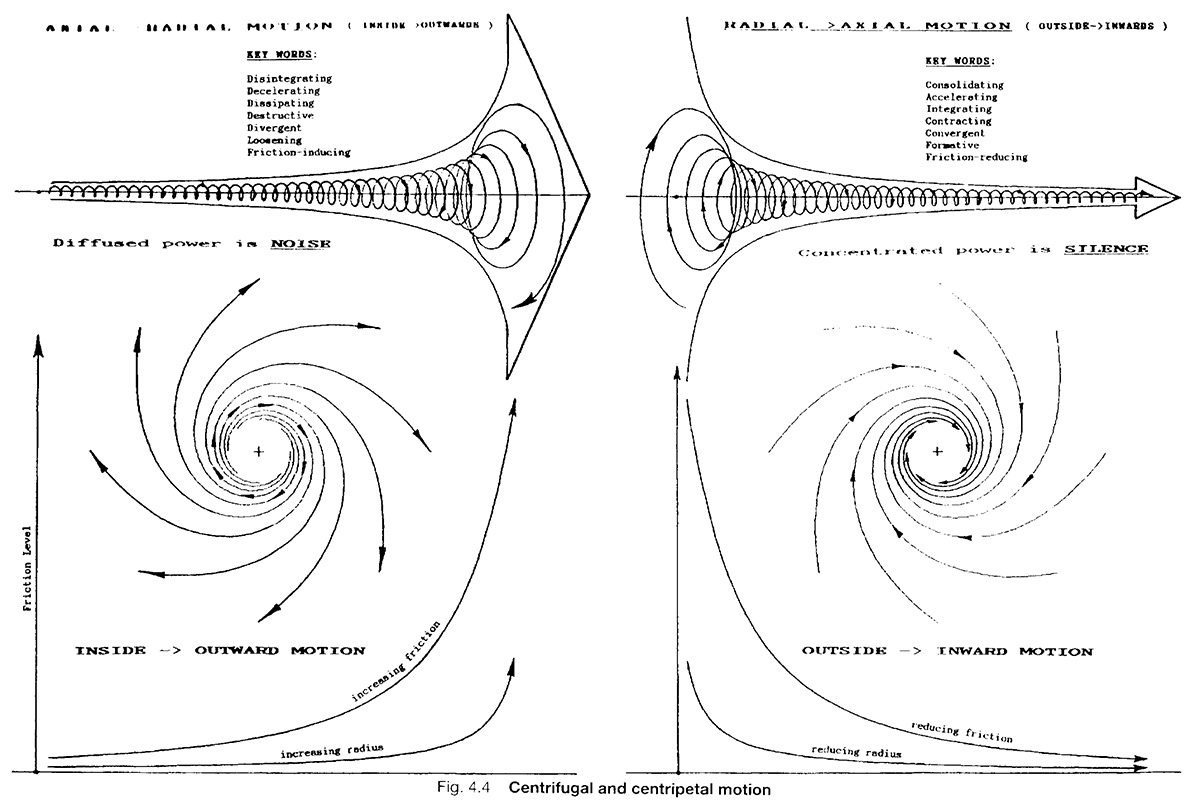
Image is the copyrighted property of Callum Coats and/or Gill Books, ©1996, 1998, 2001. From the book Living Energies, by Callum Coats. Fair use claimed for educational purposes.
Johns Hopkins University and Leonardo da Vinci both showed that the heart turns laminar blood flow into a vortex pattern (picture water swirling down a drain). The centripetal (outside-in) acceleration of vortexing takes the flow’s momentum and concentrates that energy through the center of vessels. The coldest, densest portions of blood accelerate faster through the middle, sucking the warmer, less-dense fractions after them.
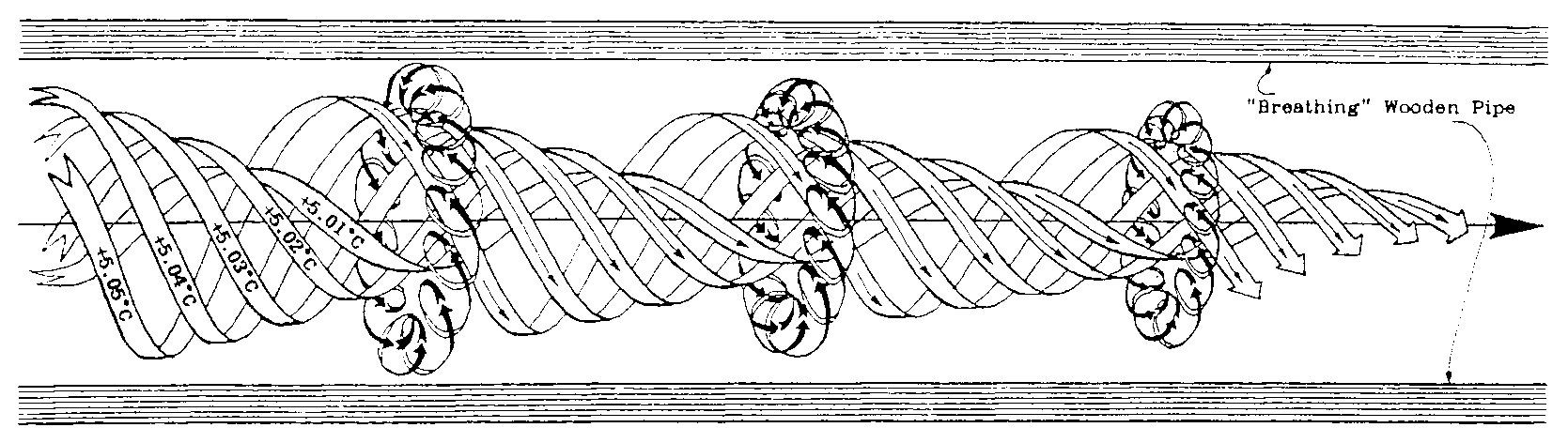
Image is the copyrighted property of Callum Coats and/or Gill Books, ©1996, 1998, 2001. From the book Living Energies, by Callum Coats. Fair use claimed for educational purposes.
The warmest fractions along the vessel wall form counter-rotating eddies, which act as ball bearings for the colder, core fractions. This flow pattern allows the warmer components of blood greater opportunity to form e-zone on the vessel walls (from IR light/heat), as well as protons, which go into the flow.
E-zone adds protons, propulsion and cohesiveness to the blood
Professor Gerald Pollack showed that when you place a hydrophilic tube in a container of water, the formation of e-zone on the inside surface of the tube releases protons into the tube. Positive polarity pushes the water out of the tube as protons try to distance themselves from each other. Water will then continue to flow into and out of the tube on its own in whichever direction the flow was initiated (or toward a wider side), powered only by light or a native electromagnetic frequency.
Similarly, Prof. Pollack theorizes in The Fourth Phase of Water that e-zone is formed on the inside of blood vessels, which would propel blood through the same mechanism as a hydrophilic tube: protons in the fluid. Blood vessels are hydrophilic tubes, after all. Subsequent investigation appears to prove Prof. Pollack correct: The interior of all blood vessels are lined with a fuzzy surface that holds e-zone in its hairs. Vink and Duling (2000) noted a 0.4-0.5 µm thick gelled layer of water lining the endothelium of all healthy vessels.
Looking somewhat like microscopic fur, the “glycocalyx,” as this layer is called, holds gel-state water which propels blood and protects endothelial cells (vessel walls) from friction. At the same time, the charge-separated water adds protons to the serum, thereby adding polarity, propulsion, and cohesiveness to the blood. Plus, propulsion from narrow to wide veins helps returning blood reach its top speed, right before it enters the heart.
Arteries employ a peristaltic “squeezing” action
Major arteries expand and contract in rhythm with the beating action of the heart. The question is, whether expansion and contraction of major vessels is a passive reaction to pressure produced by the heart, or an active driver of blood locomotion by design.
My theory is, it’s the movement of blood’s charged particles into arteries that causes their smooth muscle fibers to expand in order to pre-tension the vessels with pressure, thus storing potential energy. When the artery is full, its muscles relax and the vessel passively constricts, thereby creating a pumping action.
Think about it: Passively stretching vessels would consume the blood’s momentum that’s leaving the heart. It would waste energy. That’s not efficient. Nature would not design blood flow across the entire animal kingdom to be anything less than 100% efficient. Inconceivable.
On the other hand, programmed expansion, then automatic contraction, of vessels is way more efficient at sucking blood into vessels, then pushing it out. Never forget, we are electrical beings first, then chemical or mechanical in nature after that.
To summarize, I think the vessels do more of the pumping than commonly believed, while the heart does far less. It just appears as if the heart is doing all the pumping because it’s obviously doing something. So we just assumed it was doing everything, based on our biases toward classical Newtonian mechanics. We mistook the expansion and contraction of blood vessels to be incidental to cardiac ejection of blood, when constriction of vessels may be one of the blood’s main motives of movement.
As evidence, Professor Kurt Bergel of Berlin observed this vascular pumping action when he opened the top of bird eggs after a few days of incubation. He noticed that blood vessels surrounding the yolk-sac pulsated before the heart had even been formed. How it that possible?
Other researchers have seen blood flow continue in mice, rats and dogs from 15 minutes to several hours postmortem (i.e., no heartbeat). The most likely reason: Charged particles in the blood are not only negating friction, but also expanding vessels to put energy into the system (as blood propulsion).
Where does blood get the energy to return to the heart?
After slowing down to swap oxygen for carbon dioxide, energy for the blood’s return trip comes from propulsion away from the capillary bed and suction toward the heart (both electrostatic and mechanical). As we learned from Prof. Pollack, electrostatic expansion propels fluids away from areas of high proton concentration to low concentration (smaller vessels to larger ones). Like squeezing a wet bar of soap, blood flows away from the capillary bed as its protons repel each another.
As blood travels toward the heart, suction forces unite. The current progressively picks up speed as its kinetic energy aggregates into fewer vessels of larger size – similar to the way a slow wetland current turns into raging whitewater as more current passes through a narrower channel.
To add to that, Viktor Schauberger informed us that oxygenated blood has greater volume, so the propulsive effect is greatest from the lungs to the tissues. As that oxygen is dropped off to the tissues, pressure decreases. This creates higher pressure and propulsion before the capillary bed, and a slight vacuum on the way back. This helps blood get over the “hump” of the capillary bed, far from the heart.
Circulation summary
Our classic conception of blood flow conflicts with science, reason and reality. Just imagine: a high-pressure area making physical contact with a long succession of molecules, one after another like billiard balls, to push blood through 60,000 miles of tubing. There’s no way on earth that could be correct, based on logic and what blood flow actually looks like. Far too much energy would be absorbed and wasted by the very squishy RBCs, plasma, and vessel walls.
On the other hand, it’s easy to understand how the momentum from each surge of cells and fluid electrostatically drives preceding groups forward. Picture the RBCs’ momentum flooding arteries like tens-of-thousands of marathon runners leaving the starting line and staying a few feet apart the entire race. As pent-up energy at the valve is released, each burst of blood adds momentum not just to the group immediately ahead, but to every group in front of it, until those at the frontlines are peer-pressured to run through the single-cell capillaries.
To start the show, charged particles set the stage for blood flow to occur. Their job is successful when friction is virtually zero, and pulses of pressure are causing a ripple effect. Once the blood is moving effortlessly and homogeneously with the polarity of zeta potential, the physics behind pulsation and implosion raise the second act of the circulation story to a crescendo. Third, structuring water molecules with e-zone, and pumping the blood with vessel contractions, bring this story to its triumphant conclusion.
Each element is a piece to the puzzle of how the blood circulates around the body without breaking a sweat. Conversely, when an actor is unable to perform its duties, the show doesn’t usually end there. You have others who can pick up some of the slack. Adaptation protocols are called into action and the show goes on. Some of those corrective measures are outlined in Part VII:
To learn more about how zeta potential effects the blood, read the paper “Earthing (Grounding) the Human Body Reduces Blood Viscosity – a Major Factor in Cardiovascular Disease” by Chevalier, G., Sinatra, S., (2013), The Journal of Alternative and Complementary Medicine, Vol. 19, No. 2, 2013, pp. 102–110. Link here.